Biomedical Engineering Reference
In-Depth Information
Aperture
distance
measurement
To p
coil
Trans-
former
Capacitor
Bot-
tom
coil
Capacitor
Current
measurement
Source
Magnetic-
field
regulation
Remote-control
PC
FIGURE 17.14
NanoActivator
tm
with internal schematic. (From Gneveckow, U. et al.,
Medical physics
31, 20 04.)
Although the system has the capability to deliver fields up to
18 kA/m, practical field strengths have been limited in clinical
application. Significant patient discomfort resulting from hot
spots and subjective feelings of pain result in varying levels of
tolerance for different regions of the body. Tolerated fields have
typically been 3 to 5 kA/m for the pelvic region, 8.5 kA/m for the
upper thoracic region, and >10 kA/m for the head (Wust et al.
2006). Hot spots occur at skin folds, where the induced current
densities are highest, and bone interfaces, where it is expected
a phenomenon is occurring similar to RF heating at bounda-
rie, due to mismatches in electrical properties (Johannsen,
Gneveckow, Taymoorian, Thiesen et al. 2007).
the insertion of single or multiple 1 mm catheters contain-
ing fiber-optic temperature probes. Catheters are positioned
pretreatment and spatial temperature distributions are taken
by sliding the probe longitudinally along the catheter's axis
(Maier-Hauff et al. 2011).
A common and well-accepted method for describing thermal
dosimetry was proposed by Sapareto and Dewey, in which the
measured temperature-time curve is normalized to an equivalent,
cumulative time at 43°C (Sapareto and Dewey 1984). A represen-
tative temperature
T
90
, can be taken as the temperature exceeded
by 90% of the treated tumor volume, and can be used to calculate
the cumulative equivalent minutes at 43°C (CEM43), based on
the following:
17.5.1.5 real-time thermometry and Dosimetry
Real-time thermometry is critical for validation of predicted tem-
perature distributions and determining the treatment effects in
terms of the thermal equivalent dose. Unfortunately, compatible,
noninvasive thermography techniques are not available for use
with MFH. MR thermometry has demonstrated some promise
for noninvasive thermal measurement (Poorter et al. 1995), but
is ineffective in MFH due to the susceptibility artifacts created
by the high concentration, magnetic nanoparticle deposits (Wust
et al. 2006). However, some potential for noninvasive temperature
measurement has been proposed with US (Amini, Ebbini, and
Georgiou 2005) and CT (Fallone, Moran, and Podgorsak 1982)
thermography and could be developed for compatible application
with MFH. An additional technique uses the fifth and third har-
monics response of magnetic nanoparticles in a sinusoidal field to
estimate the bulk temperature, with an accuracy of 0.3°C demon-
strated
in vitro
(Weaver, Rauwerdink, and Hansen 2009).
Currently, though, minimally invasive, probe-based ther-
mometry is the only method of temperature measurement
during MFH. Temperature mapping is accomplished through
CEMt R
43
=
43
−
T
90
(17.18)
where
t
is the time spent at the temperature
T
90
and
R
is either
0.25 for T < 43°C or 0.5 for T > 43°C. This relation can be used to
calculate the thermal dose at a constant treatment temperature
or integrated across a temperature curve.
17.5.2 Clinical results
Seven clinical trials have been completed as of 2011, with two
additional studies in progress. A summary of the trials is included
in Table 17.4. Phase I trials are aimed to investigate feasibility,
toxicity, and tolerability of MFH treatments. Demonstration
of feasibility generally included homogeneous implantation of
the magnetic fluid, the capability to maintain therapeutic tem-
peratures in the treatment area, and validation of the calculated
temperature distributions. Phase II study is intended to demon-
strate efficacy and further evaluate safety. More specific results
and outcomes will be discussed in the subsequent sections.
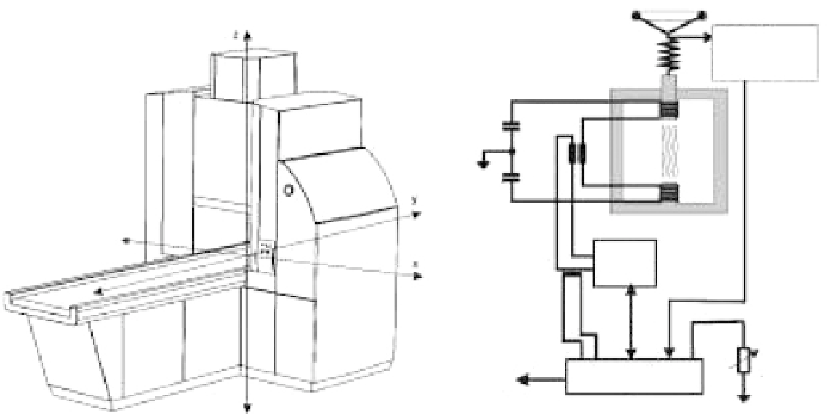