Biomedical Engineering Reference
In-Depth Information
Fig. 3.2.10-1
) is the ingrowth of tissue into pores on the
surface or throughout the implant. The increased in-
terfacial area between the implant and the tissues results
in an increased resistance to movement of the device in
the tissue. The interface is established by the living tissue
in the pores. Consequently, this method of attachment is
often termed ''biological fixation''. It is capable of with-
standing more complex stress states than type 1 implants
with ''morphological fixation''. The limitation with type 2
porous implants, however, is that for the tissue to remain
viable and healthy, it is necessary for the pores to be
greater than 50 to 150
m
m(
Fig. 3.2.10-2
). The large in-
terfacial area required for the porosity is due to the need
to provide a blood supply to the ingrown connective tissue
(vascular tissue does not appear in pore sizes less than
100
m
m). Also, if micromovement occurs at the interface
of a porous implant and tissue is damaged, the blood
supply may be cut off, the tissues will die, inflammation
will ensue, and the interfacial stability will be destroyed.
When the material is a porous metal, the large increase in
surface area can provide a focus for corrosion of the im-
plant and loss of metal ions into the tissues. This can be
mediated by using a bioactive ceramic material such as
HA as a coating on the metal. The fraction of large po-
rosity in any material also degrades the strength of the
material proportional to the volume fraction of porosity.
Consequently, this approach to solving interfacial stability
works best when materials are used as coatings or as
unloaded space fillers in tissues.
Resorbable biomaterials (type 4 in
Table 3.2.10-2
and
Fig. 3.2.10-1
) are designed to degrade gradually over
a period of time and be replaced by the natural host tissue.
This leads to a very thin or nonexistent interfacial thick-
ness (
Fig. 3.2.10-2
). This is the optimal biomaterial solu-
tion, if the requirements of strength and short-term
performance can be met, since natural tissues can repair
and replace themselves throughout life. Thus, resorbable
biomaterials are based on biological principles of repair
that have evolved over millions of years. Complications in
the development of resorbable bioceramics are (1) main-
tenance of strength and the stability of the interface during
the degradation period and replacement by the natural
host tissue, and (2) matching resorption rates to the repair
rates of body tissues (
Fig. 3.2.10-1
A) (e.g., some materials
dissolve too rapidly and some too slowly). Because large
quantities of material may be replaced, it is also essential
that a resorbable biomaterial consist only of metabolically
acceptable substances. This criterion imposes consider-
able limitations on the compositional design of resorbable
biomaterials. Successful examples of resorbable polymers
include PLA and PGA used for sutures, which are me-
tabolized to CO
2
and H
2
O and therefore are able to
function for an appropriate time and then dissolve and
disappear. Porous or particulate calcium phosphate ce-
ramic materials such as tricalcium phosphate (TCP) have
proved successful for resorbable hard tissue replacements
when low loads are applied to the material.
Another approach to solving problems of interfacial
attachment is the use of bioactive materials (type 3 in
Table 3.2.10-2
and
Fig. 3.2.10-1
). Bioactive materials are
intermediate between resorbable and bioinert. A bioactive
material is one that elicits a specific biological response at
the interface of the material, resulting in the formation of
a bond between the tissues and the material. This concept
has now been expanded to include a large number of
bioactive materials with a wide range of rates of bonding
and thicknesses of interfacial bonding layers (
Figs. 3.2.10-1
and 3.2.10-2
). They include bioactive glasses such as
Bioglass; bioactive glass-ceramics such as Ceravital, A-
W glass-ceramic, or machinable glass-ceramics; dense
HA such as Durapatite or Calcitite; and bioactive
composites such as HA-PE, HA-Bioglass, Palavital, and
stainless steel fiber-reinforced Bioglass. All of these
materials form an interfacial bond with adjacent tissue.
However, the time dependence of bonding, the
strength of bond, the mechanism of bonding, and the
thickness of the bonding zone differ for the various
materials.
It is important to recognize that relatively small changes
in the composition of a biomaterial can dramatically affect
whether it is bioinert, resorbable, or bioactive. These
compositional effects on surface reactions are discussed in
the section on bioactive glasses and glass-ceramics.
Characteristics and processing
of bioceramics
The types of implants listed in
Table 3.2.10-2
are made
using different processing methods. The characteristics
and properties of the materials, summarized in
Table
3.2.10-3
, differ greatly, depending upon the processing
method used.
The primary methods of processing ceramics, glasses,
and glass-ceramics are summarized in
Fig. 3.2.10-3
.
These methods yield five categories of microstructures:
1.
Glass
2.
Cast or plasma-sprayed polycrystalline ceramic
3.
Liquid-phase sintered (vitrified) ceramic
4.
Solid-state sintered ceramic
5.
Polycrystalline glass-ceramic
Differences in the microstructures of the five categories
are primarily a result of the different thermal processing
steps required to produce them. Alumina and calcium
phosphate bioceramics are made by fabricating the
product from fine-grained particulate solids. For exam-
ple, a desired shape may be obtained by mixing the

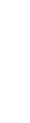



