Chemistry Reference
In-Depth Information
(a)
(b)
311
201
113
113
311
4.77
Å
113
4.11
Å
113
201
20 nm
10 nm
(c)
200 nm
FIgure 9.1
(a) Low-resolution TeM image of a monolayer of individual γ-Fe
2
O
3
nanocrystals (10.0 ± 1.5 nm). Top left: High-resolution
TeM image of one of these nanocrystals. The indicated lattice plane distances correspond to the (113) and (201) lattice planes of tetragonal
γ-Fe
2
O
3
with ordered superlattice of the cation vacancies. Top right: FFT of the high-resolution TeM image looking down the [512] zone-
axis. Adapted with permission from Ref. [19]. (b) Transmission electron micrographs (TeM) of spherical iron nanoparticles with diameters
of 2 nm. (c) electron microdiffraction pattern of rod-shaped iron oxide nanoparticles. (b) and (c) reproduced with permission from Ref. [20].
decomposition method to prepare γFe
2
O
3
, Mn
3
O
4
, and Cu
2
O nanoparticles using metal cupferron complexes as starting
materials (Me
x
Cup
x
, Me = Fe
3+
, Mn
2+
, or Cu
2+
, Cup = N-nitrosophenylhydroxylamine) (Figure 9.1a) [19].
Hyeon's group developed a similar method for the preparation of magnetic nanoparticles using iron pentacarbonyl as the
iron source and TOPO (trioctylphosphine oxide) as coordinating solvent (Figure 9.1b, c) [20]. In the following years, this
protocol was further developed to obtain high crystallinity and closer control over the final size of the nanoparticles [21, 22].
The initial methods (hot injection methods) required the swift injection of the metal precursors over preheated solvent solu-
tions, which presented several disadvantages, mainly the difficulty to scale up the processes (due to temperature inhomoge-
neities) and the risks associated with the injection over solvents at high temperatures and subsequent vigorous reactions.
Both problems were solved in 2004 with the introduction of new organometallic compounds as starting materials: oleates
and acetylacetonates. Park et al. described a very general route for the large-scale preparation of monodisperse metal oxide
nanoparticles (Figure 9.2) from oleates that were easily produced from salts of the desired metal and sodium oleate [23].
In the same year, sun and co-workers published the preparation of magnetic nanoparticles from commercial acetylaceto-
nates with high crystallinity and narrow size distribution [24]. A further advantage of thermal decomposition protocols is
their flexibility in terms of chemical nature of the nanoparticles. The methods have been used to prepare not only iron oxide
nanoparticles, but also ferrites of different nature (MnFe
2
O
4
and CoFe
2
O
4
[24], NiFe
2
O
4
[25], (Zn
x
Mn
1-x
)Fe
2
O
4
, and (Zn
x
Fe
1-x
)
Fe
2
O
4
[26]) and other types of magnetic (FePt [27-29]) and non-magnetic (Mn
x
O
y
[30, 31]) nanoparticles for MRI imaging
applications.
These protocols produce nanoparticles with optimal properties for use as MRI contrast agents. The weak point of this
methodology is that an intermediate extra step has to be introduced to produce water-soluble nanoparticles. To date, there
are only a few examples in the literature where polar high boiling point solvents have been used to prepare iron oxide nano-
crystals following thermal decomposition protocols. This advantageous modification gives a product that is directly soluble
in water, avoiding the ligand exchange step. For example, Li et al. used 2-pyrrolidone as the solvent to obtain Fe
3
O
4
nanopar-
ticles. In this case, the solvent also acts as a coating molecule, rendering nanoparticles dispersible in both acidic and alkaline
aqueous media, but not at neutral pH [32]. This methodology was further improved by using derivatised PeG molecules as
ligands to improve water solubility and at the same time provide functional groups for further modifications [33].
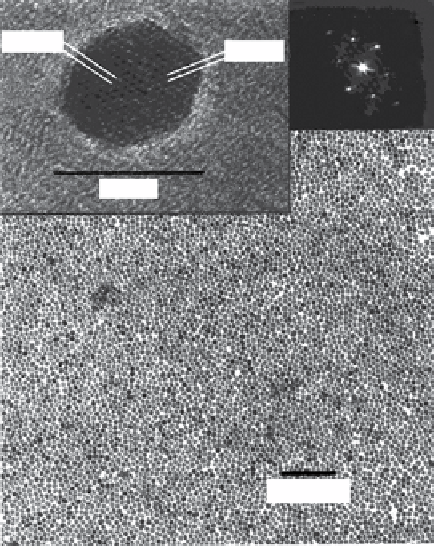
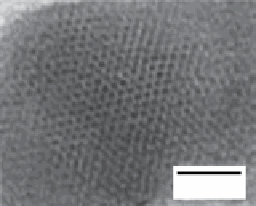
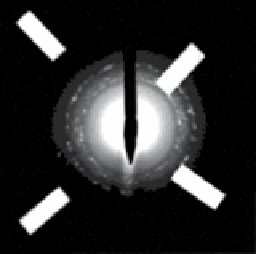