Biology Reference
In-Depth Information
protein grafting can lead to higher binding affinities in cases where the binding epitope is a
short, relatively unstructured peptide that is grafted onto a more rigid scaffold. Grafting
strategies do not necessarily require computational modeling of the system, however, as
shown by Azoitei et al.,
32
computational algorithms allow for the atomically exact modeling
of the connection between the grafted epitope and the new scaffold. In their study, the
authors took a discontinuous epitope (i.e. an epitope consisting of two backbone segments)
from the HIV protein gp120 that a broadly neutralizing antibody, b12, had been raised
against, and, after scanning a large scaffold library for complementarity to both parts of the
epitope, then transplanted both segments onto a different scaffold in the same relative
orientation. After creating a library (also guided by computational design) around the
grafted epitope, a design was obtained that bound b12 with similar affinity as the original
viral protein. In theory, when challenging the immune system with these designs, they could
raise antibodies with similar binding properties as b12, and thus this strategy could be used
to design vaccines.
In summary, several methods have been developed recently for the de novo design of
protein
protein interactions. Which method is most suited in a given situation must be
decided on a case-by-case basis. If the target of interest has certain structural features that
can be exploited to create a binding site, then approaches like beta-strand assembly or
metal-mediated interfaces can be considered. If other binders against the target protein
already exist, epitope grafting or the hotspot design method are good strategies. If neither is
available, DDMI can be used, or ligand-docking algorithms can be used to generate inputs
for hotspot design.
COMPUTATIONAL DESIGN OF CATALYTIC ACTIVITY
Of equal importance in biology as protein
protein interactions, the ability of enzymes to
accelerate chemical transformations is involved in virtually every biological process. And just
like being able to rationally engineer protein binding, being able to rationally design new or
modify existing catalytic activity would tremendously expand the synthetic biologist
110
s
horizon. Potential synthetic biology applications are the redesign of metabolic pathway
enzymes to yield novel biofuels, or the de novo design of enzymes with catalytic activities
not available in the repertoire of natural enzymes, which would allow for these
nonbiological transformations to be included in biological contexts and pathways.
'
In this section, we would like to highlight the advances made so far in the computational
design of catalytic activity. Broadly speaking, there are three types of problems in the realm
of computational enzyme design. These are, in order of increasing difficulty:
1.
Redesign of an existing enzyme active site to process a different substrate (so-called
spec-
'
).
2.
Redesign of an existing enzyme active site to catalyze a different type of chemistry (
ificity redesign
'
'
reac-
).
3.
De novo design of novel catalytic activity into a previously inert scaffold.
tivity redesign
'
Arguably, (re-)designing an enzyme is more challenging than designing a binding protein.
For the latter, it is enough to present a rigid surface that is complementary in shape to the
design target, and usually only one target needs to be recognized. An enzyme, however, at a
minimum needs to be able to bind the substrate(s) with sufficient affinity, then stabilize the
transition state with even higher affinity, but then not have too high affinity for the product.
In addition to that, often residues in the enzyme active site show large pKa shifts or form
covalent intermediates with the substrate(s), and thus the enzyme
s active site environment
needs to modulate active-site residue reactivity. This necessity to concurrently satisfy several,
sometimes conflicting criteria has led to enzymes being referred to as
'
masters of
'

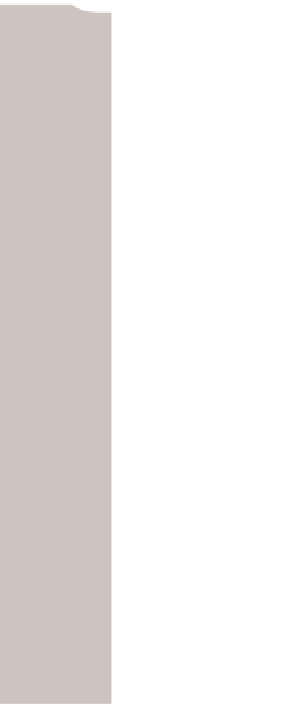