Geoscience Reference
In-Depth Information
Fig. 4.3
The balance of forces
these are different permutations—in principle related and
ultimately equivalent—to the problem of relating the forces
seen by an object to the density and speed of the fluid
relative to it. In fact, the approach we will take is based on
the aerospace convention.
It is an interesting point of trivia that the relation of force
to the density of a fluid, and the square of the velocity was
recognized theoretically by Isaac Newton, although the
subtleties of fluid mechanics are such that his idealizations
are actually most accurate for the interaction of rarified
atmospheres with spacecraft. The first practical calculations
on fluid body forces were made in 1691 by Newton's friend
and advocate, Edmond Halley. He recognized—viscerally,
from his experiences wearing a diving helmet he invented
himself and attempting salvage on the seabed—the over-
whelming role of fluid density, that forces in water running
at a given speed are 800 times higher than wind at the same
speed. Halley calculated how fast a sailing ship should
move, and even how large wings a human would need in
order to fly (Lorenz 2012).
The balance of forces on a particle (Fig.
4.3
) determine
whether it will move. First is its weight W. The effective
weight, by Archimedes' principle, is really the difference
between the vacuum weight of the particle (its mass times
the gravitational acceleration g), and buoyancy, the weight
of fluid that the particle displaces. For a sphere of diameter
d and density qp immersed in a fluid density q
f
, this is then
W=(p/6)d
3
(q
p-
q
f
).
The drag force arising from fluid motion is defined to
apply in the direction of the relative wind (i.e., opposite to
the direction of motion relative to the fluid). For a perfectly
spherical nonrotating body in a uniform flow field, drag is
the only fluid force that will act.
In general, due to irregularities in particle shape and the
gradient in fluid speed near the ground, and sometimes due
to particle rotation, the fluid force will have components
orthogonal to the fluid motion. Usually only the component
in the plane containing the fluid velocity and the
gravitational acceleration vectors is of interest—the lift
(A side-force component can exist, and is of interest in many
sports—see e.g. Lorenz 2006.). Lift manifests itself as a
reduced pressure on the upper surface of the object com-
pared with the lower surface. The application of a force to
the object (like an airplane wing) must be accompanied by a
corresponding force applied to the airstream, which is
deviated downwards as a result. The fluid may or may not
flow more quickly on the upper side of a lifting body: it is not
required to (although many textbooks make the mistake of
saying so) as an inclined flat plate can generate lift without
forcing the air over a longer path. Lift can also be produced
by rotation of the particle, the Robins-Magnus effect which
is important, for example, in lofting golf ball trajectories.
Finally, forces exist at the contact between a particle and
the surface it rests on (or between the particle and other
particles). In the high-school physics block-on-a-plane
problem, we would think of these as the normal force and
friction; between particles we might think of it as cohesion.
These forces will try to balance the others up to some
maximum value, which they cannot exceed.
If these forces are in balance, the particle will not
accelerate. If it is at rest, it will stay at rest. The weight of
the particle is the product of its volume (and thus the third
power of its diameter), its density, and gravity. Lift and drag
vary as the particle cross-sectional area (and thus the square
of diameter). Thus, all else being equal, the balance
becomes dominated by weight as diameter increases, and so
big rocks tend to sit on the ground.
The lift and drag are also proportional to the fluid density
rf, and the square of the fluid speed U. The constant of
proportionality is a dimensionless number, termed the lift
coefficient C
l
(or drag coefficient, C
d
). The lift coefficient is
a complex function of shape; for many particles it may be
close to zero, but can be of the order of 0.1. The drag
coefficient is also somewhat dependent on shape and surface
roughness, but less so than the lift coefficient. For large
objects (we will return shortly to what determines 'large'),
C
d
is often in the range 0.5-1, although it can be much
higher for very small particles, as we discuss shortly.
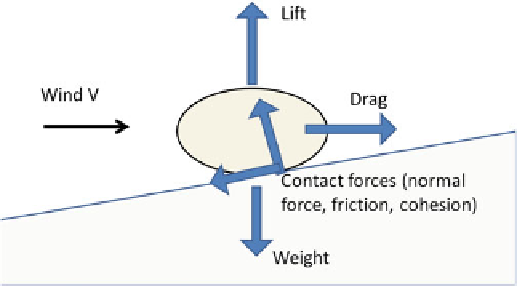
Search WWH ::

Custom Search