Geoscience Reference
In-Depth Information
stress in each layer,
W
1
and
W
2
, and the wilting point at which transpiration has declined
to zero for each layer,
W
1
and
W
2
. The proportion of roots active in transpiration can also
be divided between the two layers, adding one extra parameter. Thus, this far the model has
17 parameters for a single land cover element. It may be argued that many of these parameters
may be physically meaningful and can be estimated on the basis of soil and vegetation char-
acteristics but one of the limitations of using this type of model at large scales is knowing how
local variability should be reflected in effective values of the parameters (see Section 1.8).
An additional snow storage and melt component can be added to the model at the expense
of additional parameters (Andreadis and Lettenmaier, 2006). Liang
et al.
(1994) also show how
the model can be applied separately to each of a number of different land cover classes. Land
cover classes vary in their leaf area index, aerodynamic resistance, architectural resistance,
minimum canopy resistance, soil layer storage capacities and relative fraction of roots in the
two soil water stores. Increasing the number of land cover classes therefore means that more
parameter values must be specified.
The model is completed by routing of the fast runoff production and the baseflow to the
catchment outlet. This was originally carried out using two linear storage elements with different
mean residence times, that for the baseflow being longer than that for the fast runoff. These
parameters are currently difficult to estimate from physical measurement or reasoning and are
generally calibrated by comparison of observed and predicted discharges. An improved runoff
algorithm for the VIC model has been implemented by Liang and Xie (2001).
As well as the link back in time to the Stanford Watershed model noted above, there are
some interesting similarities between features of this model and others to be discussed in
Chapters 4, 5 and 6.
This model contains the following assumptions:
A1 Infiltration storage capacity is distributed in space according to a power law distribution.
A2 Vertical recharge to the lower store is calculated as gravity drainage depending on storage
in the upper layer store using a Brooks-Corey hydraulic conductivity function.
A3 Drainage from the lower layer store is calculated from a function that has both linear and
nonlinear segments.
A4 Calculated actual evapotranspiration takes account of wet and dry canopies, storage in
upper and lower soils, and the proportion of active roots in each store.
A5 Runoff generation from the upper store and baseflow drainage from the lower store are
routed through parallel linear stores with different time constants.
A6 Different vegetation types may be modelled separately, regardless of their spatial distri-
bution in the catchment (and at the expense of introducing more parameters).
Box 2.3 Control Volumes and Differential Equations
An easy way of understanding the development of the type of differential equations that are
used in the physically based descriptions of surface and subsurface flow processes is through
a control volume approach. Here, we start by considering the mass balance equation for a
one-dimensional channel flow, with
x
representing distance in the downstream direction and
t
representing time. We define a control volume of length
x
and look at the changes that take
place over a small time step
t
(see Figure B2.3.1). Over the time step, the change in storage
in the control volume depends on the net balance of the input from upstream
Q
i
, the lateral
recharge into the channel per unit length of channel
q
(which might be negative if there is
infiltration into the channel bed), and the output discharge from the control volume
Q
o
.
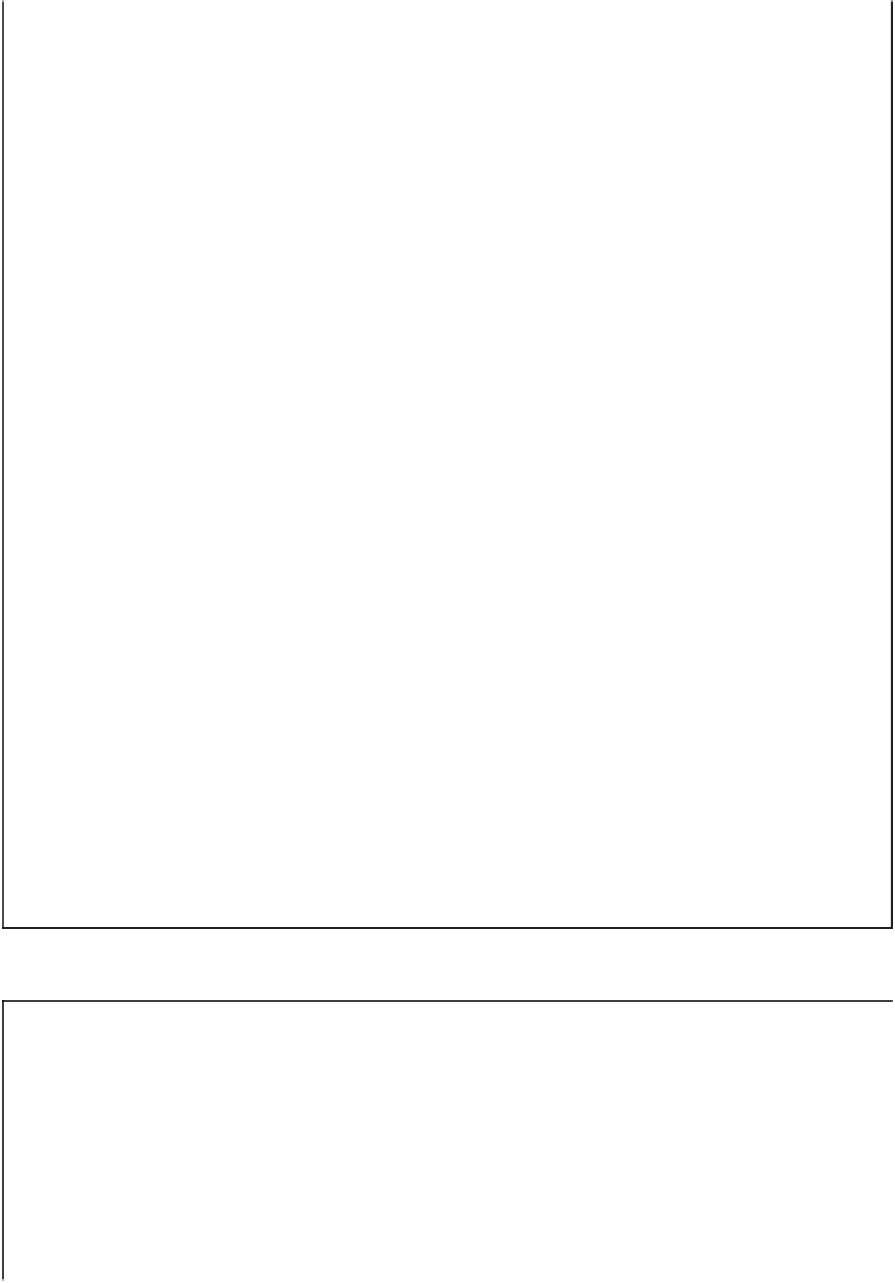