Geoscience Reference
In-Depth Information
Maslowski
, 2008;
Francis and Hunter
, 2006, 200;
Shimada
et al.
, 2006;
Perovich
et al.
, 200]. A better understanding of
these mechanisms and their relationship to increasing green-
house gas concentrations is an important step for assessing
future predictions of Arctic climate change.
Numerous studies indicate that the atmospheric circula-
tion played an important role in driving Arctic sea ice de-
clines from the 1960s to the early 1990s [e.g.,
Deser
et al.
,
2000;
Rigor
et al.
, 2002;
Hu
et al.
, 2002;
Rigor
and Wallace
,
2004;
Rothrock and Zhang
, 2005;
Stroeve
et al.
, 200;
Ser-
reze and Francis
, 2006;
Ukita
et al.
, 200]. In particular, the
declines during this period were due in part to a trend in the
dominant pattern of wintertime atmospheric circulation vari-
ability over the high-latitude Northern Hemisphere known
variously as the “North Atlantic Oscillation,” “Arctic Oscil-
lation,” or “Northern Annular Mode” [
Hurrell
, 1995;
Deser
,
2000;
Thompson and Wallace
, 2000], collectively referred
to hereinafter as the “NAM.” In particular, the anomalous
cyclonic wind circulation associated with the upward trend
in the winter NAM flushed old, thick ice out of the Arctic
via Fram Strait, causing the winter ice pack to thin, which,
in turn, preconditioned the summer ice pack for enhanced
melt.
Since the early 1990s, however, the trend in the NAM has
reversed sign, yet Arctic sea ice has continued to decline
[
Overland and Wang
, 2005;
Comiso
, 2006;
Serreze and
Francis
, 2006;
Maslanik
et al.
, 200;
Serreze
et al.
, 200;
Stroeve and Maslowski
, 2008]. This has led to speculation
that the Arctic climate system has reached a “tipping point”
whereby strong positive feedback mechanisms such as those
associated with ice albedo and open water formation effi-
ciency are accelerating the thinning and retreat of Arctic sea
ice [e.g.,
Lindsay and Zhang
, 2005;
Holland
et al.
, 2006].
These positive feedback mechanisms leave the ice pack
more vulnerable to forcing from other processes, natural and
anthropogenic. For example, enhanced downward longwave
radiation associated with increases in air temperature, water
vapor and cloudiness over the Arctic Ocean [
Francis and
Hunter
, 2006] along with enhanced ocean heat transport
into the Arctic [
Polyakov
et al.
, 2005;
Shimada
et al.
, 2006;
Stroeve and Maslowski
, 2008] and positive ice-albedo feed-
back [
Perovich
et al.
, 200] have become dominant factors
driving summer sea ice extent declines since the mid-to-late
1990s. There is also evidence that the winter atmospheric
circulation has continued to affect the winter sea ice distri-
bution since the mid-1990s [
Comiso
, 2006;
Maslanik
et al.
,
200;
Francis and Hunter
, 200].
The purpose of this study is to revisit the issue of Arctic
sea ice trends from 199 to present in the context of evolving
atmospheric circulation conditions. In addition to examining
trends over the entire period of record, we investigate trends
over the two halves separately as a simple way of character-
izing their evolution. We note that the first half coincides
with an upward trend in the NAM, while the second half
coincides with a downward trend. We are particularly in-
terested in assessing the evolving role of thermodynamic
atmospheric circulation forcing of sea ice concentration
trends, taking into account any seasonal dependencies. We
use 5-day running mean sea ice concentration data on a 25
km × 25 km grid derived from passive microwave meas-
urements from 1 January 199 through 31 October 200.
Early results were presented by
Deser and Teng
[2008] for
the winter and summer seasons only.
Our study is organized as follows. Section 2 describes the
data sets and methodology. Section 3.1 provides results on
trends in Arctic sea ice extent throughout the annual cycle,
as well as derived quantities such as the timing of the sea-
sonal cycle. Section 3.2 presents the spatial patterns of sea
ice concentration, sea level pressure, and wind-induced at-
mospheric thermal advection trends for the two halves of
the study period and for the record as a whole, stratified by
season. Air temperature, sea surface temperature (SST), and
net surface downward longwave radiation trends from 199
to present are also shown. Section 4 provides a summary and
discussion of the results.
2. DATA AND METHODS
Daily sea ice concentrations (SIC) on a 25 km × 25 km
grid for the period 1 January 199 to 31 October 200 were
obtained from the National Snow and Ice Data Center. These
data are derived from the Nimbus Scanning Multichannel
Microwave Radiometer and Defense Meteorological Satel-
lite Program (DMSP) F8, F11, and F13 Special Sensor Mi-
crowave/Imager radiances using the NASA team algorithm
[
Cavalieri
et al.
, 1999].
In addition to daily SIC, we use monthly mean sea level
pressure (SLP), 1000 hPa zonal and meridional wind com-
ponents, 2-m and 1000-hPa air temperatures on a 2.5º × 2.5º
latitude grid from the National Centers for Environmen-
tal Prediction/National Center for Atmospheric Research
(NCEP/NCAR) reanalysis project [
Kalnay
et al.
, 1996] for
the period January 199 through October 200. We also use
monthly mean sea surface temperature data from the Had-
ISST1 data set [
Rayner
et al.
, 2003] on a 1º × 1 º latitude
grid, updated through December 2006. The NCEP/NCAR
reanalysis and HadISST1 data sets were obtained from the
Data Support Section at NCAR. Finally, we make use of
daily net surface downward longwave radiative fluxes de-
rived from the NASA-NOAA Television and Infrared Ob-
servation Satellite (TIROS) Operational Vertical Sounder
(TOVS) polar pathfinder data set [
Francis and Hunter
,
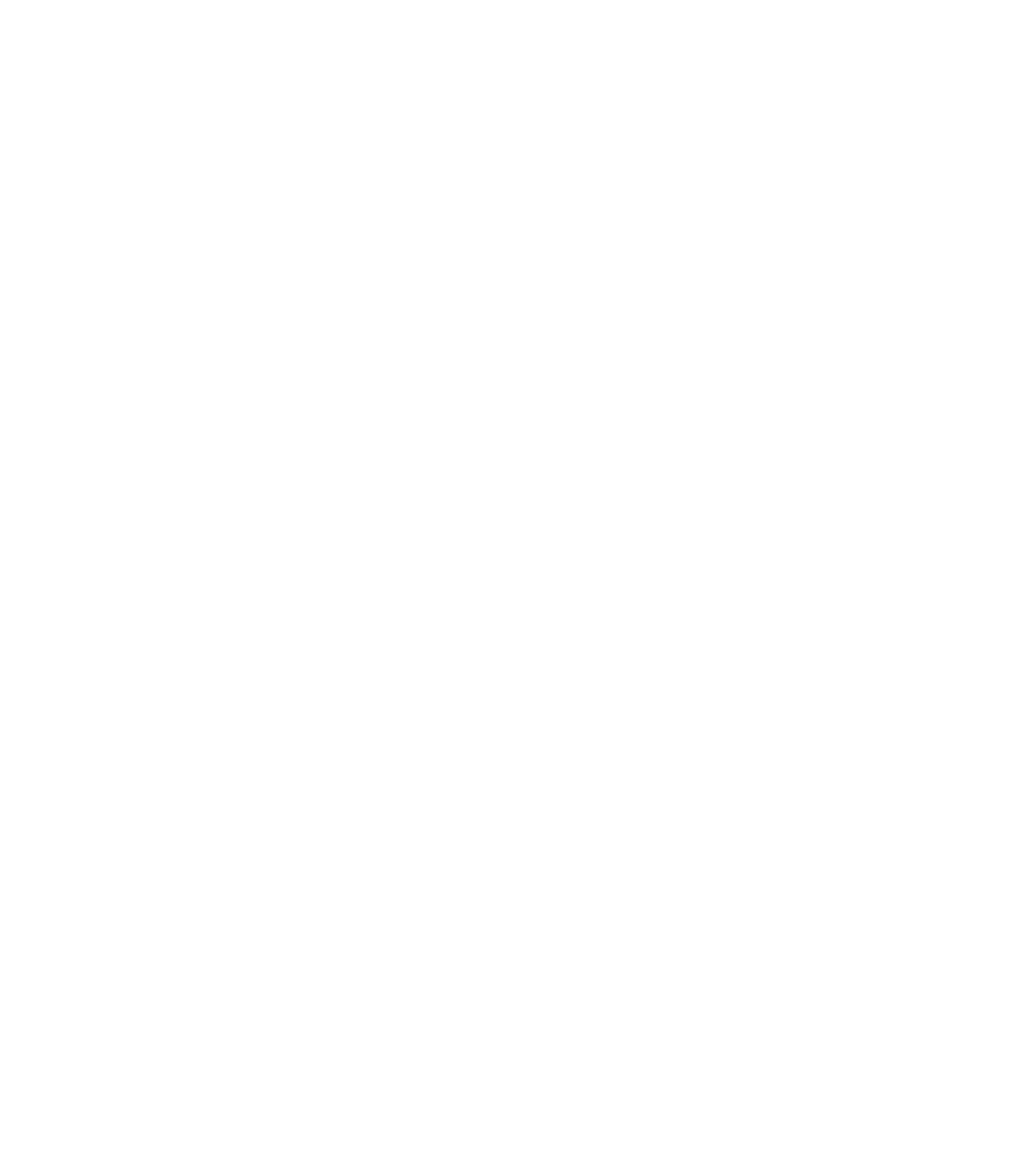
Search WWH ::

Custom Search