Environmental Engineering Reference
In-Depth Information
0
these estimates can be for steady or nonsteady
water flux.
The theory of heat flow in geologic media
and its relation to groundwater systems is
presented in a number of texts (Bear,
1972
; de
Marsily,
1986
; Domenico and Schwartz,
1998
;
Ingebritsen
et al
.,
2006
) and is briefly described
here. Water vapor movement, evaporation, and
condensation are important heat transport
processes in geothermal reservoir engineering
(Ingebritsen
et al
.,
2006
) and in diffuse water
movement through unsaturated zones of arid
regions (Philip and de Vries,
1957
; Scanlon and
Milly,
1994
; Walvoord
et al
., 2002a, b; Constantz
et al
.,
2003
). However, for the purposes of this
text and for using heat as a tracer, most recharge
studies are concerned only with the movement
of liquid water. So water-vapor transport will
not be addressed herein.
The heat-flow equation for single-phase
water movement (within the temperature range
for liquid water) is given by Healy and Ronan
(
1996
):
Autumn
Spring
1
Winter
Summer
2
3
10
15
20
25
30
Temperature (°C)
Figure 8.1
Hypothetical soil-temperature profiles at
four times during a year (reprinted from
Fundamentals of
Soil Physics
, Hillel (
1980
), Figure 12.4, copyright (1980), with
permission from Elsevier).
energy exchange between the atmosphere and
the soil surface. Parsons (
1970
) distinguished
between two zones in the subsurface: the
sur-
ficial zone
, where temperatures fluctuate over
time in response to energy exchange at the
surface, and the deeper
geothermal zone
that is
largely isolated from the influence of climate.
Within the geothermal zone, heat flows at a
relatively steady rate toward the surface as indi-
cated by a vertical profile (called the
geothermal
proile
or
geothermal gradient
) of increasing tem-
peratures with depth, typically at rates of 0.01 to
0.02°C/m. The depth to which the surficial zone
extends varies. Short-term energy exchanges
at land surface result in measurable tempera-
ture changes to depths of 0.1 to 0.3 m on a daily
basis and to about 10 m on an annual basis
(Anderson,
2005
). The magnitude of diurnal
or seasonal fluctuations decreases with depth
from land surface (
Figure 8.1
). Water move-
ment affects temperature profiles within both
the surficial and geothermal zones. Within the
geothermal zone, a temperature-depth profile
at a single point in time can be used to gener-
ate an estimate of steady, vertical water flux. In
contrast, estimates of water flux within the sur-
ficial zone usually rely on tracking changes in
surface and subsurface temperatures over time;
∇⋅
[ ()
K
θθ
+
∇ −∇ +
=∂ +1−
CD
]
T
CqT
q CT
*
*
T
w
w
w
H
(8.1)
[
θ
C
(
φ
) ]/
CT
∂
t
w
s
where
K
T
is thermal conductivity, θ is volu-
metric moisture content,
C
w
is heat capacity of
water,
D
H
is the hydrodynamic dispersion ten-
sor,
T
is temperature,
q
is specific flux (drain-
age rate, if vertical flow is assumed),
q
* is flux
from a source or to a sink at temperature
T
*,
ϕ is porosity, and
C
s
is heat capacity of the dry
rock matrix. The two primary mechanisms
for heat flow within the subsurface are con-
duction and advection; these are represented
by the first and second terms, respectively, on
the left-hand side of Equation (
8.1
). Conductive
heat flow is the movement of heat (or energy)
from areas of higher temperature to areas of
lower temperature, a mechanism analogous
to molecular diffusion. Advective heat flow
is the transport of heat due to water move-
ment. For example, water, with a temperature
of
T
1
, infiltrating and percolating through an
unsaturated zone where initial temperatures
were less than
T
1
, is a source of heat to the
subsurface. Distinguishing the advective com-
ponent of heat transport from the conductive
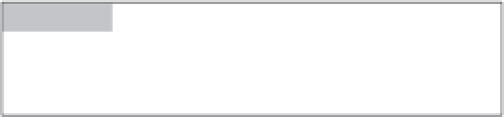
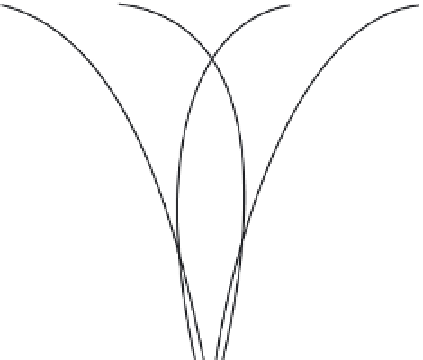
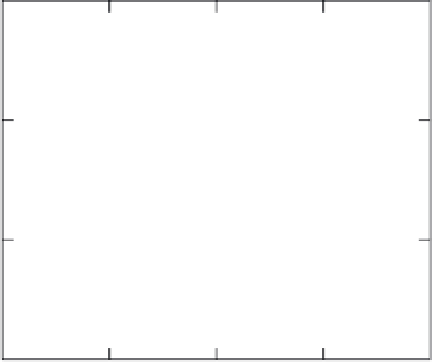
