Geoscience Reference
In-Depth Information
7.3.2 Description of the numerical
experiment
We use the volumetric water content given in Figure 7.5,
the VSP velocity data given in Figure 7.7, and the addi-
tional material properties given in Table 7.1 to construct
the water saturation, electrical resistivity, and seismic
velocity distributions shown in Figure 7.11. The area of
interest corresponds to a 25 m× 25 m 2D subsurface
model that matches the seismic split-spread acquisition
(Figure 7.12b) and incorporates the seismoelectric
and borehole VSP geometry. We place a seismic
source on the top surface at position S
0
(0 m, 0 m). The
seismic source is a Gaussian function with a magnitude
of 120 Nm that typifies a hammer blow on a plate
(Keisswetter & Steeples, 1995), and the dominant
frequency is
f
c
=500 Hz. Simulating the geometry of the
field experiment, the numerical experiment used 24
geophones in split-spread configuration (Figure 7.6)
and four electrodes making up two sets of electrical
dipole receivers (Figures 7.5 and 7.6a). The separation
between the geophones is 1 m, and the two electrodes
for each dipole receiver are spaced 2 m apart at 0.5 m
and 2.5 m distances from and in-line with the shot point.
We solve the poroelastodynamic wave equations and
the Poisson equation for the electrical potential in the fre-
quency domain using COMSOL Multiphysics 4.2a. We
compute the poroelastic and electric property distribu-
tions given the porosity, fluid permeability, and satura-
tion profiles, before solving for the displacement of the
solid phase and the pore fluid pressure. Then, we
compute the electrical potential by solving the Poisson
equation coupled to the poroelastodynamic problem
for a frequency range of 10
1000 Hz that is characteristic
of seismic and seismoelectric measurements. We then
compute the inverse fast Fourier transform (FFT
−
1
)to
get the time series of the seismic displacements,
u
x
and
u
z
, and the time series of the electrical potential response
(see Jardani et al., 2010). The meshing option for the
whole computation is 0.25 m × 0.25 m grid squares that
are smaller than smallest wavelength of the seismic
wave, which corresponds to the smallest mesh for which
the solution of the partial differential equation is mesh
independent. At the four external boundaries of the
domain, we apply a 2.5 m thick convoluted perfectly
matched layer (C-PML; see Chapter 4 for further details
on the implementation).
-
7.3.3 Model application and results
The application of this numerical model follows a four-
step strategy (steps I to IV as described below):
Step I. We compute the full 24-channel seismograms
and electrograms (Figure 7.12), dominated by the seismic
direct wave as well as two seismic reflections (annotated
in Figure 7.12a) that correlate with major changes in
VSP-derived seismic velocities centered at depths of ~4
and ~7 m (Figure 7.7). The associated electrogram is
characterized by corresponding coseismic returns from
the direct wave and the two reflections and by a series
of seismoelectric conversions with a direct field contribu-
tion (annotated in Figure 7.12b).
Log(resistivity, Ohm m)
Permeability (m
2
)
Water content (—)
m
2
× 10
−15
0.32
0
9
0.3
2.6
8
5
0.28
7
2.5
0.26
6
10
0.24
2.4
5
0.22
4
2.3
15
0.2
3
0.18
2.2
20
2
0.16
1
2.1
12.5
-12.5
0
12.5
-12.5
0
12.5
-12.5
0
Distance (m)
Distance (m)
Distance (m)
(a)
(b)
(c)
Figure 7.11
Material properties distribution constructed for modeling.
a)
Permeability.
b)
Volumetric water content.
c)
Electrical resistivity distribution. In addition, we use a constant porosity for the domain of interest (Table 7.1). (
See insert
for color representation of the figure
.)

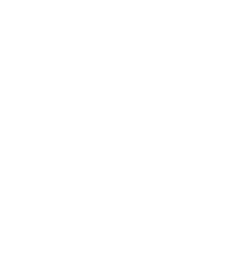








































































































